7. A Benchmark for Compact CO2 Capture Plant Designs by Monoethanolamine Solvent Testing at Technology Centre Mongstad (2021)
Gelein de Koeijera, Christophe Benquetbc, Anette Knarvikac, Erik Gjernesd, Steinar Pedersena, Odd Arne Hvidstenac
aEquinor ASA, P.O. Box 8500, 4035 Stavanger, Norway b Total E&P Norge, Finnestadveien 44, Dusavik, 4029 Stavanger, Norway cTechnology Centre Mongstad, 5954 Mongstad, Norway dGassnova SF, Dokkvegen 10, 3920 Porsgrunn, Norway
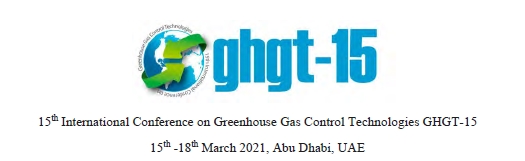
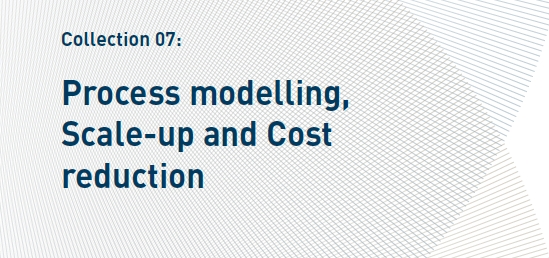
Technology Centre Mongstad (TCM) is a large and flexible demonstration site for post-combustion CO2 capture. It is located next to the Equinor refinery at Mongstad (Norway) which is the source of the flue gases supplied to TCM. It has been used for testing CO2 capture with MEA solvent and a compact design, providing a benchmark for compact CO2 capture technologies.. The absorber was used with its lowest possible packing height of 12m, and only one of two water washes was used (3m height). The plant was operated with a high flue gas flow rate (67,000 Sm3/h) and 35 wt% MEA with a sensitivity down to 30 wt%. The CO2 inlet concentration was 6% mimicking some industrial exhausts, small turbines with exhaust gas recycle or modern turbines with high turbine inlet temperatures. The tests demonstrated that such a low absorber can capture more than 80% of the CO2 with only a slightly higher steam demand than conventional applications with higher packing heights of 18-24m. The low absorber gave 3.9 MJ/kg CO2 for the specific reboiler duty, while previous tests at other conditions and with higher absorbers gave
3.5-3.7. Acceptable emissions were observed, while degradation was higher than earlier TCM campaigns due to the choice of running with 35 w% MEA. Overall, a benchmark has been provided for future improved compact capture technologies.
Technology Centre Mongstad (TCM) is a large and flexible demonstration site for post-combustion CO2 capture. It is located next to the Equinor refinery at Mongstad (Norway) which is the source of the two types of flue gases supplied to TCM. These sources are the combined cycle gas turbine (CCGT) based heat and power plant (CHP) and residue fluid catalytic cracker (RFCC). TCM is owned by Gassnova (on behalf of the Norwegian state), Shell, Total and Equinor. Various proprietary amine solvents have been tested and matured at TCM since the start-up in 2012. In addition, various campaigns have been executed with a numbered series of open non-proprietary monoethanolamine (MEA). Most of the results have been published. The most significant campaigns were the third monoethanolamine test campaign (MEA-3) in June 2017 and the following MEA-4 and MEA-5 that lasted until October 2018. The large number of public, industrial, research and academic participants involved in these campaigns have enabled that the results served a broad scientific audience. The main objectives of these campaigns were to gain knowledge and information that can be used to reduce the cost as well as technical, environmental and financial risks of commercial scale deployment of post-combustion capture (PCC). This includes demonstration of a model-based control system, dynamic operation of the amine plant, investigating amine aerosol emissions, establishment of residue fluid catalytic cracker (RFCC) baseline performance with MEA, and specific tests targeted at experimentally verifying measures that can reduce the cost of CO2 avoided [1].
This paper describes a part of the MEA-5 campaign tests relevant for reducing the size of a CO2 capture plant as the most important driver. Reduced size can be beneficial or even enable CO2 capture in certain applications. One example is brownfield retrofit of capture on exhausts with very limited spaces, e.g. existing industry near urban areas and refineries. Another example are turbines or engines on offshore oil&gas production unit. Equinor is currently developing its 3CWI concept “CO2 Capture with Carbonated Water Injection” for greenfield Floating Production, Storage and Offloading (FPSO) units [2]. In this concept CO2 is captured from a gas turbine, compressed, mixed with produced and/or sea water and injected as dissolved bicarbonate ions. For these examples the main constraints is available space and equipment weight rather than the energy efficiency. As the absorber is the unit of largest mass and volume, experiments that can reduce the uncertainty at low absorber heights are of value for these applications. Not much specific research has been done yet with this motivation.
The tests in this paper are done at elevated CO2 concentration relative to earlier CHP based campaigns at TCM. 6 vol% was chosen, which reduces the size of the absorber relative to the usual 3.5-4 vol%. The reason for this is that elevated CO2 concentrations are likely in applications where weight and volume are restricted. One motivation for this choice is the possible use of exhaust gas recycle (EGR) both on turbines and piston engines. Another motivation is the gradual development of increasing the CO2 content due the higher turbine inlet temperatures in modern turbines allowing for less air cooling of the expander blades. In order to achieve 6 vol% of CO2 in the flue gas going to the capture plant, the TCM amine plant was operated with flue gas from the Mongstad CCGT plant and with recycling a portion of the captured CO2.
The absorber packing height was set at 12m, which is the lowest possible at TCM’s amine unit. Moreover, one of the two water washes was disabled. This combination simulates the lowest absorber setting possible at TCM, but has significant dry bed height in between. The results will be used for discussing the viability of making post-combustion capture more compact. It is acknowledged that the amine unit at TCM is not specifically designed for testing compact capture. Consequently, the result may not be representative for commercial use as the unit will operate outside the operational window TCM’s amine unit is designed for. The data can be utilized as a source for insight, comparison and for benchmarking commercial compact capture technologies.
TCM’s amine unit was used (see e.g. [4][5][6]) and its flexibility was utilized to collect data relevant for a compact capture plant design. This means that the absorber was operated with only the lower packing section (12 m) and with one water wash bed (3 m, Lower (L) in operation, Upper (U) is idle) in service. This is the smallest total amount of packing possible at TCM without modifications. The set-up is illustrated and compared to the full amine plant set-up in Figure 1.
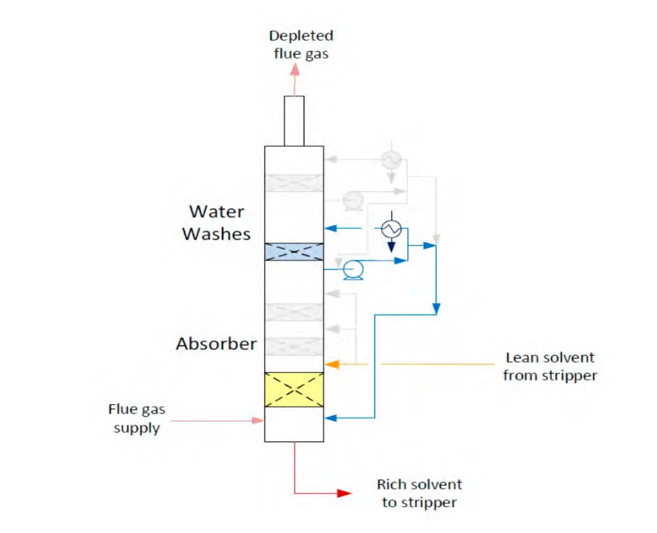
Figure 1. Illustration of the compact flue gas absorber set-up.
The flue gas flow rate was adjusted to its highest practically possible operating point (67,000 Sm3/h, 113% of design capacity). To mimic higher capacity solvent types, the MEA concentration was increased to 35 wt% from the normally used 30 wt%.
The amine plant has extensive instrumentation with multiple measurements on the streams required for performance assessment. Details on the CHP flue gas characteristics, the main systems in the plant and an assessment of instrumentation quality as well as calculation methods for the main parameters are described in previously published papers, see Gjernes et al [1][7].
For this work various test series were executed named by the first letters in the alphabet. The plant was operated with a high flue gas flow rate (67,000 Sm3/h). An overview of the test series is given in Table 1. The strategy in this work was to start out with the highest achievable capture rate in test series A. Therefore, the steam flow rate to reboiler was maximized and the solvent flow rate was high (106-138 kg/hr). The series B to D were systematic experiments to produce steam demand optimum curves (so-called U-curves) for identifying the lowest specific reboiler duty (SRD) for each series over a selected range of target capture rates (~ 83 to 72 %). In the E and F-series the flue gas temperature into the absorber was higher (45 instead of 30 °C), for assessing potential reduction in the cooling requirement and the equipment size of the direct contact cooler (DCC). As in the A series, the E cases were maximizing the steam flow rate to achieve a high capture rate and the F series was systematic experiments to find the energy optimum. The E case consists only of one test. All these series were run with around 35 w% MEA. Next, some tests were added to put the series A-F in a wider perspective. The cases named B5-1 and B5-2 are two reference runs with MEA at 30 wt% and with lower and upper water wash in operation, respectively. The motivation was to see whether there are differences between the upper and lower water washes. The case named 15-3 is included in the results section to have a comparison against the most compact absorber line-up used in the previous TCM study on modes of operation with cost saving potential back in 2018 [1]. The objectives for the experimental work were to investigate capture rates and specific reboiler duty during parameter testing. Steam flow to reboiler and solvent flow rates were the main variables, while flue gas flow and CO2 inlet concentration were kept constant.
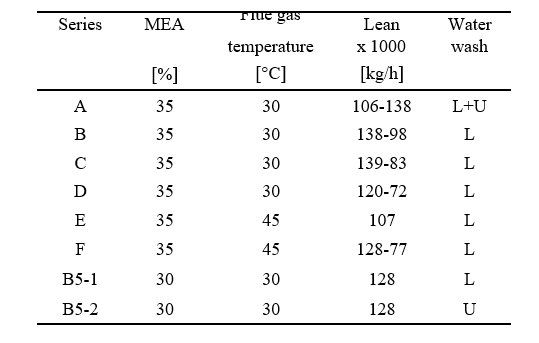
Table 1. Overview of the tests.
With this strategy, it was the intention to inspire, and stretch the targets for new technology developments of compact and low weight CO2 capture plants. With more advanced solvents and optimized systems, it is expected that significant improvements beyond what TCM demonstrates here are possible.
The so-called U-curves were produced by variations in the lean amine to gas flow rate ratio (L/G). The cold rich by-pass of the lean rich heat exchanger (17% by-pass) was in operation during all test runs. This variable was left unchanged. It is acknowledged varying it could provide somewhat lower steam demand or higher capture rate, but not significant.
The combination of methods used in the current work is shown in Table 2. The flow out of absorber is based on flow into the absorber assuming all components except water and CO2 are conserved. Moisture is calculated from temperature and pressure. The product flow is assumed to be CO2 and moisture only.
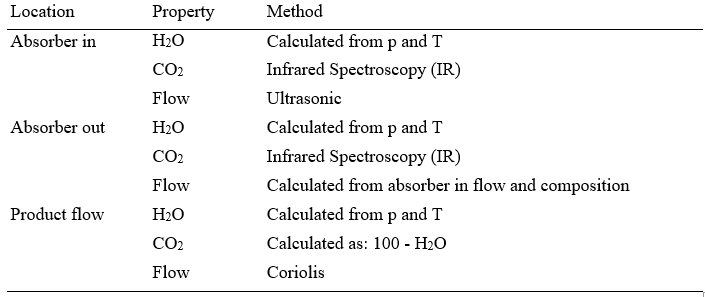
In each test run the plant is first allowed to stabilize over several hours. A set of key performance indicators is used to assess the quality of data. A two-hour stable period is selected for data extraction. Liquid sampling is conducted within this period. All properties except liquid samples (amine concentration and loading) are averaged over the two-hour period. Thus, reported data are based on stable operation with respect to all streams. The total and CO2 mass balances should be within 100 ± 2%. This procedure allows normally not more than two single test runs per day (24 hours). To make comparisons between the test runs, it is important to maintain stable MEA concentration (35 wt%, CO2 free) and the absorber inlet CO2 concentration (6 vol%, dry). MEA concentration, lean and rich loading are calculated from laboratory analysis of the liquid samples. In case there is a missing liquid sample, the lean loading is extrapolated using amine density (only for the following tests: B6-opt, C2, D4 and F2).
The solvent loading is calculated from total inorganic carbon (mole CO2/kg solvent) and total alkalinity (mole amine/kg solvent):
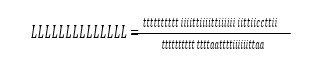
The specific reboiler duty (SRD) is the heat delivered to the reboiler from the steam flow divided by the amount of captured CO2:
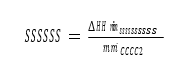
In this work the captured CO2 (𝑚𝑚̇ 𝐶𝐶𝐶𝐶2) is based on the difference in mass flow of CO2 over the absorber (𝑚𝑚̇ 𝐶𝐶𝐶𝐶2abs, in – 𝑚𝑚̇ 𝐶𝐶𝐶𝐶2abs, out). This is chosen in order to aligned with earlier published results (e.g. [1]). Captured CO2 (𝑚𝑚̇ 𝐶𝐶𝐶𝐶2) based on product flow from stripper will for the present cases result in a lower CO2 product flow. The steam pressure and temperature are typically around 2.5 barg and 160 °C in this work.
The capture rate is the mass fraction of captured CO2 and the amount of CO2 flowing into the absorber.

The absorber packing volume and thus the absorber size are major CAPEX elements. The absorber packing volume (m3/ton CO2, h) was calculated for the cases with highest CO2 product flow and compared with the previous test case 15-3. The parameter is one metric used for assessing compact absorber designs. The TCM absorber cross section is 7.2 m2 and packing height applied is 12 m.
The emissions of amine and ammonia were monitored during all test runs. As higher MEA concentration is associated with increased solvent degradation [3] and plant corrosion, degradation and metal content in the solvent were closely monitored. However longer-term testing is expected to be needed to assess the solvent management aspects of higher MEA concentrations. Moreover, the solvent composition was measured before and after for estimating the cumulative degradation.
The observed relation between SRD and capture rate is shown in Figure 2 for all the series A-F and the B5-2 and B5-1 with 30 w% MEA. The lowest SRD value in the U-curves achieved within each test series is shown as transparent squares along with a dashed trend line based on the five selected optimum points. The trend line shows a corresponding decrease in capture rate and SRD from 87% and 4.3 MJ/kg CO2 down to 73% and 3.7 MJ/kg CO2. Within each series the capture rates were quite well controlled within the target. The trend line gives a good indication of what is achievable with a compact plant configuration. The results from the F-series (82% capture rate) shows promise also for reducing cooling need and size of the DCC. It is also seen that the SRD is significantly higher for the two test runs with MEA 30wt% (B5-2 and B5-1). The uncertainty at TCM is discussed in other papers [4][5][6] and is assumed not to be significant different in this campaign.
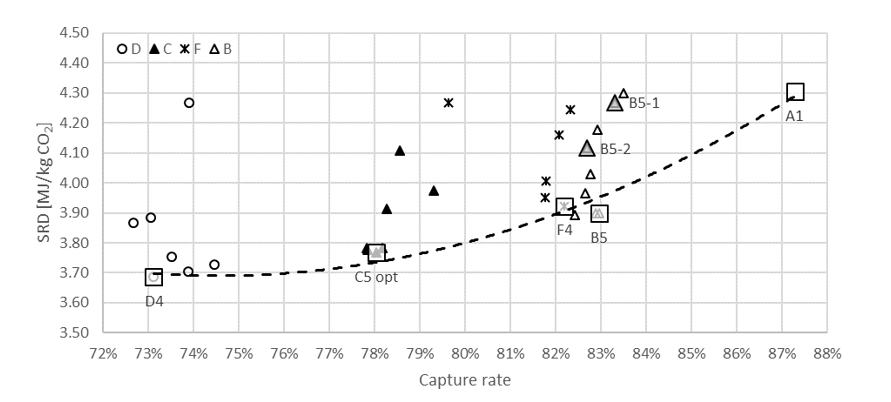
Figure 2. SRD and capture rate for the test series. Optimum points for each series are shown as transparent squares.
As expected with the low absorber height the SRD become higher than TCM has reported earlier for higher absorber heights. For 30 w% MEA Gjernes et al.[1] reports down to 3.6 MJ/kg CO2 at 86% capture with 3.6 vol% CO2 in the inlet, while this work shows around 4.0-4.2 MJ/kg CO2 at 87% capture with 6 vol% CO2 in the inlet. This increase may not be unacceptable high in situations where energy ample cheap heat is available. Figure 2 also shows that a similar low SRD of around 3.6-3.8 is achievable with a lower capture rate of 70-80%. This decrease may neither be unacceptable in the mentioned applications where space and weight are the limiting factors.
The MEA and wet CO2 inlet concentrations were aimed to be kept constant for all tests, and the achieved results are shown in Figure 3. There are small variations between the test series and the comparison between the series are representative. There is also good agreement between the two CO2 measurement methods. The figure also shows MEA concentration close to 31 wt% for the B5-1 and B5-2 cases and the CO2 concentration in good agreement with the other test series. This means that any differences are likely not to be caused by variations in CO2 inlet concentration and MEA concentration.
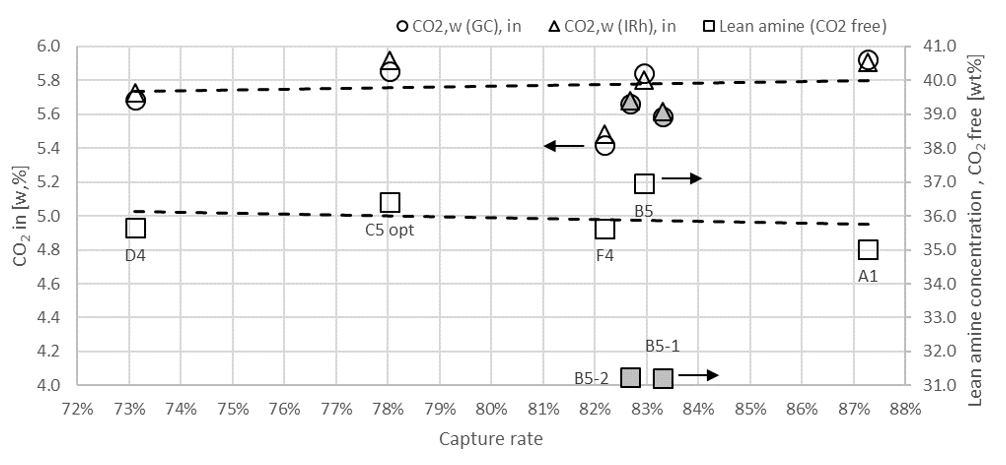
Figure 3. Wet CO2 inlet concentration and lean amine concentration were maintained at the same level for the selected optimum cases. Grey symbols are for the B5-1 and B5-2 cases that were operated at lower amine concentration.
A graph of the variations in L/G and lean loading versus capture rate is given in Figure 4. There was a wide enough experimental window with respect to lean flow and resulting loading to observe trends. A 14 percentage points higher capture rate (from 73 to 87) demands a 0.03 mole/mole lower lean loading and a 0.3 kg/Sm3 higher L/G. It is observed that the MEA 30wt% test cases (B5-1 and B5-2) needs a significantly (0.4 kg/Sm3) higher solvent flow rate to achieve the target capture rate of 82-83% than the results with 35% MEA, while the lean loading is not very different. As mentioned before, all cases are with 67,000 Sm3/h flue gas flow.
A summary of results from the test series is given in Table 3. The CO2 captured in the optimum cases shown in Figure 2 are used for calculating the absorber packing volume.
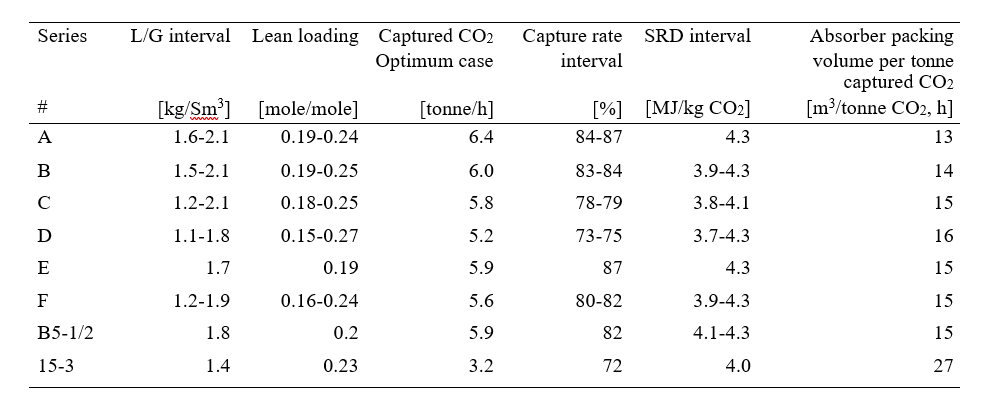
Table 3. Results summary for all test series.
The new property added in this Table is the “Absorber packing volume per ton captured CO2. This is a good property for analyzing the potential for size and weight reductions. Most interestingly, in the A-1 case the CO2 produced is 6,427 kg/h and the absorber packing volume parameter is 13. The result for the 15-3 case from earlier TCM publication
[1] was 27. This shows that there are significant differences in this property and that this campaign had low number ranging from 13-16. The difference with 15-3 up to 27 shows that there should be a significant opportunity for more compact absorber designs and corresponding cost saving for this largest equipment item in post-combustion capture. It is recommended to use this new property in future work for comparison.
A final observation is that no large differences were observed between B5-1 and B5-2. This means that there are likely no big differences between upper and lower water wash (as expected) that can explain any variation or invalidate any conclusions.
When changing design of amine based post-combustion one must always keep control of the emissions since these pose of the main HSE risks [8]. Table 4 gives an overview of the measured emissions to air at moments or intervals the results were regarded as reliable and representative. The letter gives which series the measurement is taken from while the number gives which test it is (not reported in detail in this work). Two measurements were done in the C5 test. The online data were 5 min averaged data over an analyzed period of 2 hours.
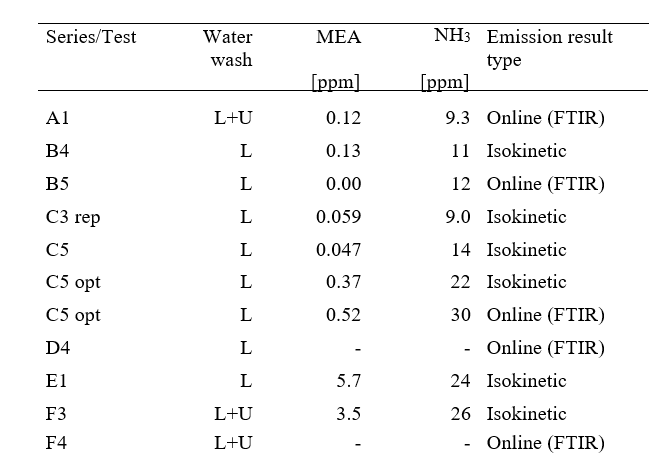
Table 4 Overview of isokinetic sampling during the period. Note that FTIR values are reported for time slots where isokinetic samplings were not available. In addition to optimum cases, emission results are included from B4, C3 rep, E1 and F3. The FTIR was out of service during case D4 and F4.
Except for E1 and F3 the emissions to air were not significantly higher than the ones published earlier on industrially representative operation [9]. In E and F especially the MEA emissions are high, probably higher than most future emission permits will allow. The reason is that the inlet temperature was increased from 30 to 45 °C. So, this seems not to be a favourable measure for compact capture. This indicates that two water washes are likely needed for compact capture if high inlet temperature is unavoidable.
The numbers presented here have a somewhat higher uncertainty than TCM presents in dedicated emission papers, e.g. Morken et al [9]. Some numbers may be somewhat higher than if the plant was operated stable for longer time. Build-up of MEA in water wash can occur. On the other hand, some numbers may be low since they can be impacted by the large amount of dry packing bed above the absorption bed. It must be noted that the quantification limit for the FTIR is 0.5 ppm due to increased instrument noise at low levels. However these uncertainties do not impact the conclusion on the overall low enough levels with 30 °C inlet and the overall too high levels at 45 °C inlet temperature.
When changing design of amine based post-combustion one must also always keep control of the degradation since it can be important for the OPEX and HSE risk. Table 5 gives an overview of metal and heat stable salt (HSS) in the solvent. The compact campaign was done after another campaign of 905 hours. So, the amine was already partly degraded at the start.
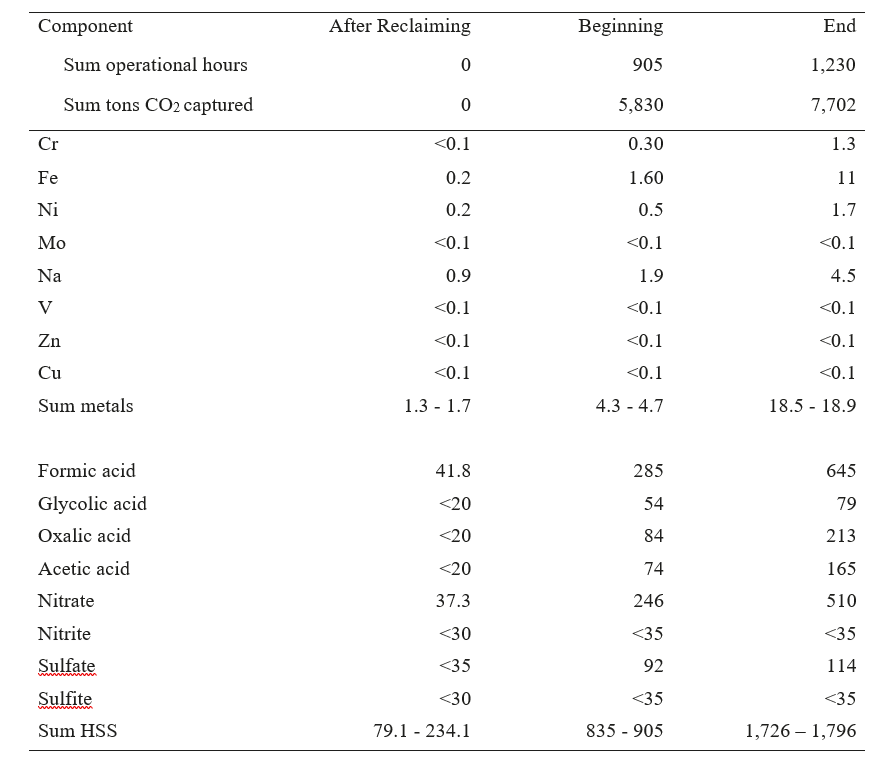
Table 5. Overview of metal and heat stable salt (HSS) concentrations after reclaiming and at the beginning and end of the campaign discussed in this paper. All concentrations are mg/kg solvent. Components below the detection limit are marked with “<”.
The degradation products increase as expected. When deciding to run this campaign with 35wt% a risk of excessive degradation was taken. This risk was mitigated by only running a relative short time of 325 hours. From all the measured concentrations the main discussion in literature focuses on the iron concentration. TCM’s own guideline
[10] recommends keeping the iron concentration below 5 mg/kg solvent. In the compact campaign it increased from from 1.6 to 11 crossing the recommended value. However, Moser [11] has observed long periods of low degradation above the 5 mg/kg iron. It seems anyhow recommended to have more mitigation actions in place for keeping the degradation under control for using 35 w%. Examples are continuous reclaiming or use any of the new O2 and/or iron removal technologies.
Over the years various campaigns have been executed at TCM with MEA on CCGT flue gas, of which the compact campaign in this paper is the latest. From each campaign one representative result was chosen that has most industrial relevance. Table 6 below gives an overview of these representative results enabling a comparison and perspective of what TCM has been achieved at TCM.

Table 6. Comparison of representative results from all MEA campaigns at TCM.
The results from this compact campaign has as expected the largest SRD as well as the lowest capture rate. The differences with the other cases on variables that are important for cost and HSE are not very large. Hence, we can conclude that the amine unit at TCM can be used to study compact capture, although it is not specifically designed for it. Another result from this Table is a set of data that can be used to tune any overall MEA model. The data give a large enough specter to serve this purpose.
The results in this paper give a benchmark for any compact capture technology. Many new ideas and improvements on reducing weight and size can use this work for comparison. Preferably, these should perform better on most variables that are important for cost and HSE. Examples of such more compact but less mature technologies are rotating absorption&desorption, membrane contactors and CO2 selective gas-gas membranes.
But better results could also be obtained at TCM in the future. One improvement idea for TCM is to use another better solvent. This work already shows that increasing the MEA concentration from 30 to 35% makes compact capture design more attractive. TCM has already tested the solvent CESAR-1 for other motivations [12]. Testing this solvent on compact design like done in this work is a logic next step.
Another idea for TCM is to study in more detail metal build-up and degradation rates in compact design. This work provides a good indication but had not enough hours for industrially relevant conclusions. A final idea is further testing at 45°C and two water washes could also be interesting to be able to find the balance with the size of the direct contact cooler (DCC) upstream the absorber and the lean amine cooler.
The amine unit at TCM has been used for testing compact capture for providing a benchmark. The absorber was used with its lowest possible packing height of 12m, and only one of two water washes was used (3m height). The plant was operated with a high flue gas flow rate (67,000 Sm3/h) and 35 wt% MEA with a sensitivity down to 30 wt%. The CO2 inlet concentration was 6% mimicking some industrial exhausts, small turbines with exhaust gas recycle or modern turbines with high turbine inlet temperatures. The tests demonstrated that such a low absorber can capture more than 80% of the CO2 with only a slightly higher steam demand than conventional applications with higher packing heights of 18-24m. The low absorber gave 3.9 MJ/kg for the specific reboiler duty for the low absorber, while the higher absorbers gave 3.5-3.7. Acceptable emissions were observed, while degradation was high due to the choice of running with 35 w% MEA. Overall, a benchmark has been provided for future improved compact capture technologies.
The authors gratefully acknowledge the staff of TCM DA, Gassnova, Equinor, Shell and Total for their contribution and work at the TCM DA facility. The authors also gratefully acknowledge Gassnova, Equinor, Shell, and Total as the owners of TCM DA for their financial support and contributions.
- Gjernes E, Pedersen S, Jain D, Åsen KI, Hvidsten OA, de Koeijer G, Faramarzi L, de Cazenove T, Documenting modes of operation with cost saving potential at the Technology Mongstad, Energy Procedia, 14th Greenhouse Gas Control Technologies Conference Melbourne 21-26 October 2018 (GHGT-14)
- Pettersen J, Nilssen OR, De Koeijer G, Approaching Zero CO2 Emissions for Future Oil and Gas Production Offshore, Abstract and presentation to TCCS-10 conference, June 2019, Trondheim, Norway
- Supap T, Idem R, Veawab A, Aroonwilas A, Tontiwachwuthikul P, Chakma A, and Kybett BD, Kinetics of the Oxidative Degradation of Aqueous Monoethanolamine in a Flue Gas Treating Unit, Ind. Eng. Chem. Res. 2001, 40, 16, 3445–3450
- Thimsen D, Maxson A, Smith V, Cents T, Falk-Pedersen O, Gorset O, Hamborg ES, Results from MEA testing at the CO2 Technology Centre Mongstad. Part I: Post-Combustion CO2 capture testing methodology, Energy Procedia, 63, 2014, 5938-5958,
- Hamborg ES, Smith V, Cents T, Brigman N, Falk-Pedersen O, de Cazanove T, Chhagnlal M, Feste JK, Ullestad Ø, Ulvatn H, Gorset O, Askestad I, Gram LK, Fostås BF, Shah MI, Maxson A, Thimsen D, Results from MEA testing at the CO2 Technology Centre Mongstad. Part II: Verification of baseline results, Energy Procedia, 63, 2014, 5994-6011
- Faramarzi L, Thimsen D, Hume S, Maxon A, Watson G, Pedersen S, Gjernes E, Fostås BF, Lombardo G, Cents T, Morken AK, Shah MI, de Cazenove T, Hamborg ES, Results from MEA Testing at the CO2 Technology Centre Mongstad: Verification of Baseline Results in 2015. Energy Procedia, Volume 114, 2017, p 1128-1145.
- Gjernes E, Pedersen S, Cents T, Watson G, Fostås BF, Shah MI, Lombardo G, Desvignes C, Flø NE, Morken AK, de Cazenove T, Faramarzi L, Hamborg ES, Results from 30 wt% MEA performance testing at the CO2 Technology Centre Mongstad, Energy Procedia, Volume 114, 2017, 1146-1157
- De Koeijer G, Talstad VR, Nepstad S, Tønnessen D, Falk-Pedersen O, Maree Y, Nielsen C. Health risk analysis for emissions to air from CO2 Technology Centre Mongstad, International Journal of Greenhouse Gas Control, 18, 2013, 200–207.
- Morken AK, Pedersen S, Kleppe ER, Wisthaler A, Vernstad K, Ullestad Ø, Flø NE, Faramarzi L, Hamborg ES, Degradation and Emission Results of Amine Plant Operations from MEA Testing at the CO2 Technology Centre Mongstad, Energy Procedia, 114, 2017, 1245- 1262
- Morken AK, Pedersen S, Nesse SO, Flø NE, Johnsen K, Feste JK, de Cazenove T, Faramarzi L, Vernstad K, CO2 capture with monoethanolamine: Solvent management and environmental impacts during long term operation at the Technology Centre Mongstad (TCM), International Journal of Greenhouse Gas Control 82 (2019) 175-183.
- Moser P, Wiechers G, Schmidt S, Monteiro JGMS, Charalambous C, Garcia S, Fernandez ES, Results of the 18-month test with MEA at the post-combustion capture pilot plant at Niederaussem – new impetus to solvent management, emissions and dynamic behaviour, International Journal of Greenhouse Gas Control, Volume 95, 2020.
- Christophe Benquet C, Knarvik A, Gjernes E, Hvidsten OA, Kleppe ER, Akhter S, First Process Results and Operational Experience with CESAR1 Solvent at TCM with High Capture Rates (ALIGN-CCUS Project). To be presented at GHGT-15 Virtual Conference: 15-18 March 2021.